Abstract
MicroRNAs (miRNAs) are small non-coding RNA molecules that regulate gene expression by binding to target messenger RNA (mRNA) molecules and promoting their degradation or blocking their translation. Parkinson’s disease (PD) is a neurodegenerative disorder caused by the loss of dopaminergic neurons in the substantia nigra. There is increasing evidence to suggest that miRNAs play a role in the pathogenesis of PD. Studies have identified several miRNAs that are dysregulated in the brains of PD patients, and animal models of the disease. MiRNA expression dysregulation contributes to the onset and progression of PD by modulating neuroinflammation, oxidative stress, and protein aggregation genes. Moreover, miRNAs have emerged as potential therapeutic targets for PD. This review elucidates the changes in miRNA expression profiles associated with PD, emphasising their potential as diagnostic biomarkers and therapeutic targets, and detailing specific miRNAs implicated in PD and their downstream targets.
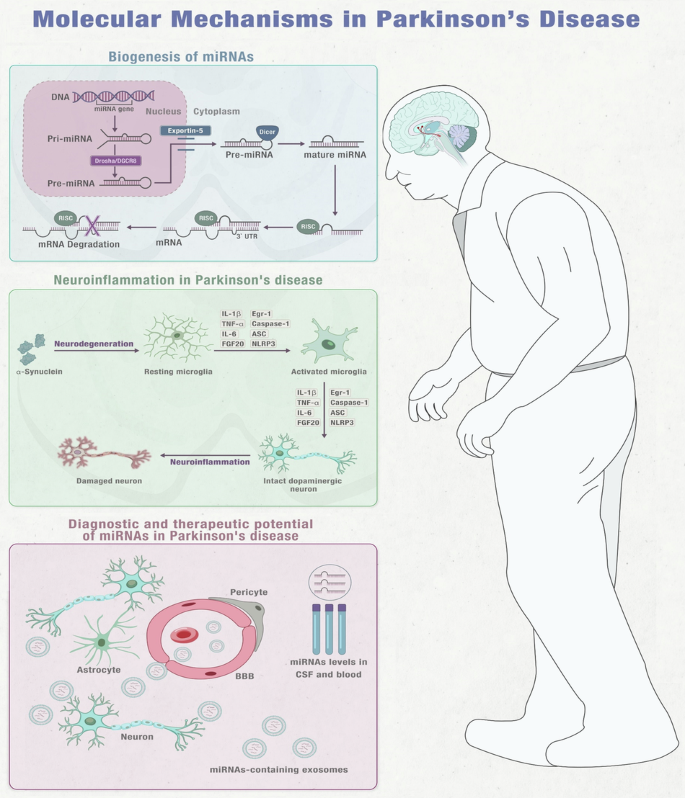
Integrated Insights into miRNA Function, Microglial Activation, Diagnostic, and Treatment Prospects in PD Note: This figure is an original figure created by the authors.
Introduction
Parkinson’s disease (PD) is a prevalent movement disorder that affects the ageing population, with an estimated prevalence of 1% in individuals over 60 years old. It is characterised by a gradual loss of neurons in the brain, particularly the dopamine-producing neurons in the Substantia Nigra Pars Compacta (SNpc) region. This results in a decrease in dopamine neurotransmitter production and signalling, leading to motor dysfunction symptoms like tremors, slow movements, stiffness, and instability in posture1,2. The fundamental mechanism behind PD is linked to disruptions in protein balance due to the misfolding and clustering of alpha-synuclein (α-Syn) proteins. This leads to the development of Lewy bodies and Lewy neurites, which are intracellular aggregates3.
Presently, the clinical treatments for PD primarily aim to alleviate symptoms and include both pharmacological options like Levodopa, dopamine agonists, catechol-O-methyl transferase inhibitors, and monoamine oxidase B inhibitors, as well as non-pharmacological interventions like deep brain stimulation4,5. While these treatments help to relieve the motor symptoms of PD, they do not provide neuroprotection, cannot halt or slow disease progression nor reverse neurodegeneration6. Both environmental and genetic factors, such as mutations in the alpha-synuclein (SNCA) and leucine-rich repeat kinase 2 (LRRK2) genes, have been implicated in the development of PD through their regulation of mitochondrial function in dopamine-producing neurons7,8. The blood-brain barrier (BBB) compromise and infiltration of peripheral immune components have also been reported in PD patients. Immune components that infiltrate the brain can interact with dopamine neurons, leading to their decline9,10. The pathological hallmarks of PD include widespread α-synuclein aggregation and the gradual degeneration of the nigrostriatal pathway, resulting in the death of dopamine neurons in the SNpc. This process ultimately manifests in both motor and non-motor symptoms associated with PD11,12,13.
Recent groundbreaking discoveries have shed light upon the pivotal role of miRNAs, dynamically governed by genes implicated in PD susceptibility, in shaping the pathogenesis of this debilitating disorder. Remarkably, accumulating evidence indicates that miRNAs exert their profound impact on PD pathophysiology by assuming a direct regulatory role in key cellular processes, specifically within mitochondrial and immune pathways9,10. Recent discoveries show that miRNAs, which are regulated by genes associated with PD risk, can contribute to the disease by directly regulating mitochondrial and immune pathways9,10.
Several studies indicate that dysregulated miRNA expression is involved in the onset and progression of PD14,15,16,17,18,19,20,21,22. Patients with PD have aberrant miRNA levels in both their brain tissue and peripheral blood16,17,19,22,23. Of particular interest are several miRNAs that target genes involved in dopaminergic neurotransmission, mitochondrial function, immune response, and SNCA aggregation through several pathways9,10,17,18.
In this review, we are going to focus on miRNA expression in PD, their biomarker and therapeutic potential, specific miRNAs and targets, and the challenges of using miRNAs therapeutically.
Biogenesis of miRNAs
MiRNAs were first discovered in 1993 and research has shown them to be crucial biological molecules that play a role in maintaining normal cellular functions24,25,26. MiRNAs are non-coding RNA molecules that come from miRNA genes and intronic sequences. They are initially transcribed as primary miRNAs (pri-miRNAs) and stem-loop precursor miRNAs (pre-miRNAs) in the nucleus. The Drosha/DGCR8 complex processes pri-miRNAs into pre-miRNAs, which are then transported out of the nucleus by Exportin-5. In the cytosol, Dicer cleaves pre-miRNAs to create double-stranded mature miRNAs. The single-stranded mature miRNA, which is usually around 20-22 nucleotides long, is then loaded onto Argonaute proteins to form the RNA-induced silencing complex (RISC). The mature miRNA attaches to complementary sequences, typically located at the 3′-UTR of the mRNA and works as a potent gene expression regulator. The targeting specificity is determined by the seed sequence located at the miRNA’s 5′-end, spanning bases 2-827,28,29 (Fig. 1)30,31.
Emerging research presents an intriguing perspective, suggesting that miRNAs within exosomes may be relatively scarce. Instead, the majority of circulating miRNAs are associated with non-vesicular extracellular particles (EPs) and extracellular vesicles such as circulating lipoproteins and other protein complexes30,31. This notion challenges the prevalent concept of positing exosomes as the primary source of miRNAs for intercellular signalling and biomarker discovery32. However, countervailing studies have furnished compelling evidence demonstrating the presence of copious and functionally active miRNAs within exosomes, capable of modulating the gene expression and phenotypic traits of recipient cells33,34. Consequently, discerning the relative contribution and biological significance of miRNAs hosted in exosomes versus Eps, the setting of PD, entails a multifaceted consideration of factors such as particle origin, isolation methods, comprehensive particle characterisation, cell type, physiological and pathological context, among others30.
Exosomal miRNAs: key players in neurodegenerative disease pathogenesis and therapeutics
Exosomes carry an array of materials like proteins, lipids, and noncoding RNAs, such as miRNA, long noncoding RNA (lncRNA), and messenger RNA (mRNA)35,36. Upon release, exosomes navigate to specific destinations, like immune cells in the central nervous system (CNS), where they exert varied effects. These vesicles are pivotal in intercellular communication, influencing disease development in immunological disorders, tumorigenesis, and neurodegenerative disorders37. They stem from sources like human mesenchymal stem cells, immune cells, and microglia, significantly impacting the progression of neurodegenerative diseases by influencing gene expression and cellular processes38,39,40. Exosomal miRNAs play a role in managing oxidative stress, a significant aspect of neurodegenerative events. Their ability to breach the BBB and concentrate in brain tissue suggests their potential as drug carriers and biomarkers for neurodegenerative diseases41,42. Additionally, their effectiveness as non-invasive biomarkers for disease progression is noteworthy15,16,17,18,19,20. Present methods for isolating exosomes encompass various techniques like ultracentrifugation, density gradient centrifugation, size exclusion chromatography, exosome precipitation, and immunoaffinity capture. Exosome characterisation involves approaches like western blotting and ELISA. These diverse methods possess distinct merits and drawbacks in terms of efficiency, purity, yield, cost, and time requirements. However, a consensus agreement on the optimal technique for both isolation and characterisation of exosomes remains elusive43,44,45,46.
The interaction between exosomal miRNAs and oxidative stress in the context of PD
The brain needs a substantial oxygen supply, and a considerable portion of this is converted into (ROS)47. Normal cellular processes, including oxidative phosphorylation in mitochondria, produce ROS like hydrogen peroxide (H2O2), superoxide anion (O2-), and nitric oxide. While these ROS are crucial for redox signalling and other cellular functions, they can also cause oxidative damage. This damage includes lipid peroxidation in cell and organelle membranes, protein oxidation through cross-linking, fragmentation, and carbonyl group formation, as well as oxidation of DNA and RNA within cells48. Antioxidative mechanisms increase the production of several antioxidants to mitigate these radicals. However, in PD, the endogenous antioxidants needed are inadequate, leading to unchecked ROS generation. This can result in the excessive accumulation of non-physiological and toxic levels of ROS, leading to oxidative stress49.
One proposed explanation posits those neurodegenerative diseases manifest through the distinct processes of apoptosis/necrosis and neuronal cell dysfunction, leading to compromised motor or cognitive functions. The CNS, due to its elevated metabolic rate and significant lipid content, is notably susceptible to oxidative stress. Elevated levels of oxidative stress are frequently observed in the brains of patients afflicted with neurodegenerative conditions50,51,52.
In PD patients, excessive ROS production elevates oxidative stress. Evidence indicates that dopamine metabolism, elevated levels of iron and calcium in the substantia nigra (SN), mitochondrial dysfunction, and neuroinflammation all contribute to the heightened oxidative stress and loss of dopaminergic neurons observed in the brains of PD patients49.
Neurodegenerative diseases are closely linked with both oxidative stress and exosome-derived miRNAs42. Interestingly, oxidative stress can influence the expression of various miRNAs, while miRNAs can also regulate many genes that are involved in the response to oxidative stress. Therefore, the relationship between oxidative stress and miRNA networks is complex and interconnected during the process of neurodegeneration42. Oxidative stress exerts a substantial impact on the expression levels of numerous miRNAs, and reciprocally, miRNAs play a pivotal role in the regulation of a multitude of genes associated with the oxidative stress response53. The interplay between oxidative stress and miRNA networks extends its influence beyond the primary regulatory axis, influencing critical processes implicated in neurodegeneration. Notably, these interconnected pathways contribute to mitochondrial dysfunction, disruption of proteostasis, and heightened neuroinflammation, collectively culminating in the outcome of neuronal death53. This intricate web of interactions underscores the comprehensive involvement of oxidative stress and miRNA networks in the multifaceted landscape of neurodegenerative processes53.
Recent research indicates that miR-34a plays a significant role in the neurotoxic pathways associated with neurotoxins like paraquat, rotenone, and 6-hydroxydopamine (6-OHDA) in PD54. However, lithium chloride, which is a mood-stabilising medication, can safeguard SH-SY5Y cells from paraquat-induced neurotoxicity by inhibiting miR-34a and activating the antioxidant protein expression regulator nuclear factor 2-related factor 2 (NRF2)55. The significance of miRNA is highlighted by the fact that Schisandrin B, an antioxidant found in dibenzo cyclooctadiene lignin, was able to reverse the inhibition of NRF2 and the increase in miR-34a expression caused by 6-OHDA in cells. This effect was observed by Ba et al.56 who also found that the behavioural improvement caused by Schisandrin B was hindered by miR-34a overexpression in a 6-OHDA PD-mouse model. Additionally, astrocytes may secrete more miR-34a under stressful conditions. In the context of PD, the function of miRNA (miR-34a) was observed through its transmission from astrocytes to dopaminergic neurons via exosomes. This transmission resulted in an increased susceptibility of the neurons to neurotoxins by regulating the Bcl-2 gene57. A recent investigation has demonstrated that increasing the expression of miR-34a may help to reduce neuronal apoptosis caused by oxidative stress. This finding underscores the importance of miRNA in this process58. Currently, our understanding of the impact of this effect on the development of PD is limited. However, based on studies conducted on cellular and animal models, it appears that miR-34a may have a pathophysiological function in PD58.
MiR-137 is a miRNA that is highly conserved. It is mainly present in the brain of Drosophila and has been observed to increase in the early stages of PD in flies. Similar results have been found in the plasma of PD patients. In a recent study, Jiang and colleagues demonstrated that reducing the levels of miR-137 in exosomes led to an increase in the expression of oxidation resistance 1 (OXR1), which provided neuroprotective effects against oxidative stress in a PD-mouse model59,60,61.
As a highly conserved miRNA, Let-7 has been extensively studied for its involvement in various physiological processes and diseases. Its dysregulation can have significant implications for human health. Specifically, Let-7 is upregulated in the PD-mouse model, suggesting a potential therapeutic target for this debilitating condition62,63. A previous study indicated that an increased level of Let-7, which is carried by exosomes, can be detected in the cerebrospinal fluid(CSF) of individuals with PD. This suggests that these miRNAs can be transported via exosomes. If neurons absorb Let-7 that is carried by exosomes, it can trigger neurodegeneration by activating toll-like receptor 7 (TLR7)64. The Let-7 group has been found to have a positive impact on the effects of functional mutations of leucine-rich repeat kinase 2 (LRRK2), which plays a role in the development of PD. In a PD-mouse model using C. elegans, the inhibition of Let-7 leads to a slight rise in ROS levels, which promotes neuronal autophagy and decreases the buildup of α-synuclein protein. This, in turn, lessens the progression of PD. These findings emphasise the significance of miRNA in the development of PD65,66.
Dysregulation of miRNAs in neurodegenerative diseases
The multifaceted involvement of miRNAs in neurodegenerative diseases stems from various mechanisms, each impacting distinct facets of neuronal function and disease progression. Firstly, miRNAs exert influence by targeting mRNA transcripts of regulatory-related genes, impeding protein translation or inducing protein degradation. This regulation significantly affects crucial aspects of neuronal biology, including survival, differentiation, synaptic plasticity, and axonal guidance. Notable instances include miR-9’s targeting of REST, a repressor of neuronal genes, fostering neuronal differentiation67. Similarly, miR-124’s interaction with PTBP1, a splicing factor inhibiting neuronal gene expression, enhances neuronal identity68. Furthermore, miR-29’s action on BACE1, an enzyme involved in amyloid beta production, mitigates amyloid beta levels69.
Secondly, miRNAs play a pivotal role in modulating neuroinflammation by directly engaging toll-like receptors or regulating their mRNA expression. This regulatory process influences the innate immune response and the production of pro-inflammatory cytokines within the brain. For instance, miR-146a’s binding to TLR4 and TLR2 curtails NF-kappaB activation, consequently dampening the expression of inflammatory genes and mitigating neuroinflammation70. Conversely, miR-155’s targeting of SOCS1, a negative regulator of cytokine signalling, amplifies the inflammatory response71.
Finally, disruptions in miRNA biogenesis contribute significantly to disease pathogenesis. Mutations or dysregulation in key enzymes involved in miRNA processing, such as Dicer, Drosha, and RISC components, disrupt miRNA formation. For instance, mutations in TARDBP, encoding TDP-43—a constituent of RISC—disrupt miRNA processing, contributing to amyotrophic lateral sclerosis68. Dysregulation of Dicer expression alters miRNA profiles, impacting neuronal function and survival in AD and PD. These multifaceted mechanisms underscore the intricate and diverse roles of miRNAs in neurodegenerative diseases, offering potential avenues for targeted therapeutic interventions.
Dysregulation of miRNAs in PD
Dysregulation of miRNAs has been linked to the development of diseases, including brain disorders and cancers72,73,74,75. In PD, research has indicated that the expression profile of miRNAs is dysregulated and may contribute to the pathogenesis of the disease76.
Numerous miRNA molecules have been discovered in immune cells, exceeding 100, which regulate the development and operation of innate and adaptive immune responses. For instance, certain miRNAs like miR-9, miR-21, miR-29b, and miR-34a are responsible for regulating MG during the innate immune response. MiRNAs, being small molecules with biologically active functions, can easily pass through the BBB77,78,79,80. This leads to the hypothesis that miRNAs could form a significant component in the construction of the secondary network within the central-peripheral inflammatory network, thus serving as a key pathway for communication between the central and peripheral inflammatory states.
In patients with PD, research indicates that miRNA expression profiles are disrupted in striatal brain tissue and dopamine neurons in the substantia nigra pars compacta (SNpc)20,81. Examining post-mortem prefrontal cortex samples from miRNA profiles of PD patients has shown significant changes in 125 miRNA molecules compared to neurologically normal controls. This suggests that miRNA dysregulation may play a role in PD pathogenesis by influencing various genes and proteins linked to the disease82 (Table 1).
MiRNAs and microglia in PD
MiRNAs play roles in PD pathogenesis by modulating inflammatory responses. Dysregulation and downstream targets of specific miRNAs, such as miR-124, miR-195, miR-let-7a, miR-150, miR-330, miR-7116-5p, miR-7, miR-190, miR-29c, and miR-30e, have potential therapeutic targets in attenuating neuroinflammation and microglial activation in PD.
MiR-124
According to Yao et al.83, miR-124, brain-specific miRNA, was significantly reduced in the MPTP-induced PD-mouse model and could suppress neuroinflammation during PD development. The researchers aimed to understand the mechanisms underlying the ability of miR-124 to inhibit neuroinflammation and discovered that the expression of p62 and p-p38 was considerably increased in the MPTP-induced PD-mouse model and BV2 cells induced with LPS. Therefore, miR-124 targets p62 and p38 to reduce MG inflammation in PD. Knocking out p62 in BV2 cells has been shown to prevent cell apoptosis and death in the SH-SY5Y human neuroblastoma cell line following MG activation. Additionally, providing miR-124 exogenously can decrease p62 and p-p38 expression and reduce MG activation in the SNpc of MPTP-induced PD-mouse model, implying that miR-124 suppresses neuroinflammation in PD development through targeting p62 and p3884. In addition, the introduction of miR-124 from an outside source can impede the production of mitogen-activated protein kinase 3 (MEKK3) and p-p65 in the SNpc of the MPTP-induced PD-mouse model. This also helps to diminish the activity of microglia, leading to the proposal that miR-124 may have the potential as a target for therapy in regulating the inflammatory response associated with PD84.
MiR-195
The role of miR-195 in modulating inflammation during the development of PD is significant. MiR-195 expression was observed to decrease in BV2 cells induced with LPS, while upregulation of miR-195 led to inhibition of the release of proinflammatory cytokines such as iNOS, TNF-α, and IL-6 while inducing the release of anti-inflammatory cytokines such as IL-4 and IL-10. MiR-195 acts by negatively regulating Rho-associated kinase 1 (ROCK1), which is responsible for inducing MG activation. The decreased expression of ROCK1 also has a similar effect on MG regulation as the overexpression of miR-195. The interaction between miR-195 and ROCK1 is crucial in inducing the activation of MG24.
MiR-let-7a
MiR-let-7a has been identified as a potential contributor to decreasing PD symptoms by acting as a negative regulator of MG-induced neuroinflammation. Previous research has revealed that the activation of the Signal transducer and activator of transcription-3 (STAT3) occurs simultaneously in the SNpc. Meanwhile, miR-let-7a targets STAT3, and the PD-mouse model showed a decrease in miR-let-7a expression. Increasing miR-let-7a expression has been demonstrated to impede BV-2 MG activation and reduce the production of pro-inflammatory agents instigated by α-syn. However, the influence of miR-let-7a was nullified by reintroducing the STAT3 protein85.
MiR-150
In the pathogenesis of PD, miR-150 has been linked to neuroinflammatory processes. The AKT signalling pathway is widely recognised for its involvement in various pathophysiological mechanisms, including the advancement of PD. According to Li et al.85, the level of miR-150 expression in PD patients was lower, and the study demonstrated that overexpressing miR-150 in BV2 cells induced with LPS cells can reduce the release of TNF-α, IL-1β, and IL-6. Moreover, the study showed that AKT3 was directly targeted by miR-150 in BV2 cells, and the overexpression of miR-150 could help treat PD as it suppressed neuroinflammation by targeting AKT386.
MiR-330
MiR-330 was found to be upregulated in two animal models, namely an LPS-induced MG chronic neuroinflammatory model and a PD-mouse model. Its overexpression induced the expression of Arg1 and SHIP1, inhibited the translocation of NF-κB, repressed M1 polarisation, and decreased iNOS expression. MiR-330 negatively regulates NF-κB activity through the MG SHIP1 target protein, thereby continuously repressing MG polarization triggered by LPS in vitro and in vivo. This provides a promising neuroprotective approach for PD treatment. SHIP1 is a downstream target molecule of miR155-5p, one of the most crucial miRNAs responsible for a robust inflammatory response. Lowering miR155-5p expression leads to upregulated SHIP1 expression and decreased NF-κB activity, thereby inhibiting inflammation and MG activation87.
MiR-7116-5p
He et al. 88 illustrated the crucial function of miR-7116-5p in MG-activated inflammation in the MPTP-induced PD mouse model. The study discovered that miR-7116-5p was suppressed and its target was TNF-α. Additionally, the study found that the amplification of miR-7116-5p expression was able to impede the development of TNF-α and the activation of MG, and it could avert the degeneration of dopaminergic neurons88,89.
MiR-7
A promising approach for treating PD is to understand how miR-7 and the NLRP3 inflammasome are regulated. According to Junn et al.89 the expression of miR-7 decreases in individuals with PD. MiR-7 has been identified to target α-syn in dopamine neurons, and it is linked to the development of PD. Zhou Y. et al.90 discovered that NLRP3 is another gene that is targeted by miR-7. In vitro analysis revealed that the transfection of miR-7 inhibits the activation of NLRP3 inflammasomes in MG, whereas the administration of anti-miR-7 aggravates the activation of inflammasomes. In the MPTP-induced PD-mouse model, the stereotactic injection of miR-7 mimics into the striatum reduced the degeneration of dopaminergic neurons and improved the activation of MG. In the pathogenesis of PD, there is a direct correlation between miR-7 and NLRP3 inflammasome-mediated neuroinflammation90.
MiR-190
In BV2 cells induced with LPS, miR-190 levels are decreased. However, increasing miR-190 expression has been found to decrease the release of pro-inflammatory factors, such as TNF-α, TGF-β1, iNOS, and IL-6. Research has established a negative regulatory relationship between miR-190 and NLRP3, and in the MPTP-induced PD mouse model, miR-190 was found to decrease neuronal damage and inhibit inflammation by negatively regulating the expression and activation of NLRP391.
MiR-29c
MiR-29c has also been found to exhibit anti-inflammatory properties in animal and neuronal models of PD, where its overexpression suppresses pro-inflammatory cytokine release, along with NF-κB and TXNIP/NLRP3 inflammasome activation. In this context, miR-29c may represent a promising target for treating PD by regulating NLRP3 inflammasomes by targeting NFAT5 and decreasing the inflammatory response of MG92.
MiR-30e
Beforehand, research indicated a significant reduction in miR-30e in the SNpc of the MPTP-induced PD mouse model. The application of miR-30e agomir decreased α-syn protein expression and abated the elevation of COX-2, TNF-α, and iNOS inflammatory cytokines. Additionally, miR-30e aimed at NLRP3, which resulted in the inhibition of NLRP3 mRNA and protein expression, leading to the suppression of NLRP3 inflammasome activation and the preservation of dopamine neurons93. (Table 2 and Fig. 2)
When neurons are damaged or dying in PD or toxin-induced models, they release impair-associated molecular patterns (IAMPs) and α-synuclein, which activate microglia through pattern recognition receptors (PRRs). This activation leads to the release of proinflammatory cytokines such as IL-1β, IL-6, TNF-α, and other inflammatory mediators including ASC, IFN2, iNOS, and Caspase-1. The release of these cytokines further activates other resting microglia, which ultimately leads to neuroinflammation or neurodegeneration in the affected area. MPTP (1-methyl-4-phenyl-1,2,3,6-tetrahydropyridine), IL-1β (interleukin-1β), IL-6 (interleukin-6), IFN2 (type 2 interferon), iNOS (inducible NOS), TNF-α (tumour necrosis factor-α), and ASC (apoptosis-associated speck-like protein containing a CARD). Note: This figure is an original figure created by the authors.
Potential clinical applications of miRNAs in conditions of PD
MiRNAs as a promising approach for gene therapy
The exosome transports active miRNAs, making it a promising alternative to virus-based gene therapy. This endogenous carrier has shown the potential to deliver miRNAs, which can be taken up by recipient cells without eliciting an immune response94. Additionally, exosomes cross the BBB, making them an ideal carrier for miRNAs compared to conventional RNA interference methods95. Furthermore, the animal model has demonstrated the potential of utilising exosomes as a delivery system in the treatment of miRNA96. The expression of miRNA varies in several neurodegenerative diseases, and certain miRNAs have been identified as playing a role in the advancement of these diseases. The use of exosomes to regulate these miRNAs is a promising avenue for gene therapy97,98. (Fig. 3).
MiRNAs as a novel method of intercellular communication dependent on the function of miRNA
Chemical receptor-mediated events are widely recognised as the primary means of intercellular communication. However, the discovery of exosome transport between cells has expanded our knowledge of this process. MiRNAs, which are a crucial component of this cargo, play a significant role in facilitating intercellular communication99,100. MiRNAs play an important role in transferring functional information at the paracrine level, as evidenced by exosomal miRNAs released from cancer cells that can influence the tumour microenvironment containing various cells such as cancer-associated fibrosis and pericytes. Furthermore, research has shown that neurons can transport miRNAs through exosomes to astrocytes, indirectly regulating the protein expression of astrocytes101,102.
The elucidation of this novel exosomal miRNA transfer mechanism between neurons and astrocytes holds profound implications for both the physiology and pathophysiology of the CNS. It has the potential to impact the maintenance and plasticity of neuronal networks103. Furthermore, this discovery unveils promising avenues for the development of biomarkers and therapeutics for neurological disorders52,103.
The current body of research demonstrates that neurons are capable of transporting miRNAs via exosomes to astrocytes, thereby indirectly influencing the protein expression of astrocytes103,104. For instance, a study identified the secretion of neuron-specific miR-124-3p by neurons in exosomes, which were subsequently internalised by astrocytes. This internalisation resulted in the downregulation of GFAP, a protein integral to astrocyte activation104. Additionally, another study revealed that exosomes derived from astrocytes exhibited differentially expressed miRNAs under pathological conditions such as neuroinflammation and oxidative stress. These miRNAs were implicated in potentially modulating neuronal morphology and synaptic transmission103.
Given the crucial function of astrocytes in the CNS, it is not unexpected that they have been linked to the development and advancement of neurodegenerative illnesses. The aforementioned findings indicate that neurons might influence astrocyte protein expression by emitting miRNA-containing exosomes, which could be involved in the pathogenesis of neurodegenerative conditions. This could pave the way for further research on the correlation between exosomal miRNA and neurodegenerative diseases.
MiRNAs as biomarkers for PD
Early detection of neurodegenerative diseases is crucial, but also difficult due to pathological changes occurring years prior to symptom onset. As exosomes are natural carriers of miRNAs and cross the BBB, miRNA expression is known to be altered in various neurodegenerative diseases. It is, therefore, reasonable to suggest that exosomal miRNAs in peripheral blood may vary across different diseases. To explore this possibility, exosomal miRNAs were analysed in blood samples from patients with Parkinson’s and Alzheimer’s diseases105,106. Earlier research has examined the exosomal miRNA profiles found in the CSF of individuals with Parkinson’s and Alzheimer’s disease. These studies revealed notable changes in the miRNAs when compared with those found in healthy individuals. In the future, as miRNA profiles continue to be analysed and data from various expressions are integrated over time, exosomal miRNAs have the potential to become a valuable biomarker for identifying neurodegenerative diseases107,108.
Molecular markers are substances that are produced by cells and can be continuously detected in body fluids, tissues, or cells. Exosomal miRNAs are an example of molecular markers found in body fluids, which remain stable and intact due to their protection from degradation by ribonuclease (RNase). In addition, these miRNAs can be safely stored for up to 48 hours at 4 °C in vitro, making them an excellent option for research and clinical applications. The role of micro-RNA as molecular markers is essential for understanding the diagnosis and treatment of various diseases109. The favourable properties of exosomal miRNAs aid in the quality of samples tested, highlighting their potential clinical use as biomarkers for specific diseases. Numerous research works have explored the use of miRNAs as biomarkers for neurodegenerative ailments109,110,111,112.
Gui et al. found that during the initial stages of PD, there were 27 exosomal miRNAs originating from the CSF of patients with anomalous expressions. Among them, miR-153, miR-409-3p, miR-10a-5p, and Let-7g-3p were markedly raised, whereas miR-1 and miR-19b-3p were significantly lowered. This suggests that these miRNAs could be promising biomarkers for early detection of PD. These results further emphasise the critical role of micro-RNA in identifying and treating PD107. Moreover, it is believed that decreased levels of miR-19b and elevated levels of miR-24 and miR195 in serum exosomes could be indicative of PD in patients. Furthermore, a different investigation has proposed that miR-331-5p and miR-505 in plasma exosomes could be useful biomarkers. However, these findings are not uniform, similar to research on Alzheimer’s disease105,113.
Several miRNAs showed increased expression in plasma, including miR-155–5p, which normalised after treatment and could potentially serve as a marker for disease progression monitoring114. Moreover, four miRNAs (let-7 g-3p, miR-10a-5p, miR-153, and miR-409–3p) consistently showed higher levels in exosomes from CSF115. Conversely, miRNAs such as miR-146a-5p114, miR-1, miR-19b-3p115, and miR-133b14 were downregulated, with miR-34b and miR-34c showing reduced expression in early PD stages116. Modulating these miRNAs is proposed as a strategy for PD treatment. Additionally, miR-7 depletion was noted in PD-affected regions117. The current literature only provides weak, but promising evidence about circulating miRNAs supporting their potential as biomarkers, including specific miRNA profiles identified in PD patients and the importance of larger cohort studies for validation. (Table 3).
Challenges in miRNA research in the field of PD
Multiple candidate miRNAs have been identified that might influence the progression of PD either through their effects within the CNS or in peripheral tissues. miRNAs can modulate numerous signalling pathways because each miRNA has the potential to target and bind to an average of 100–200 genes, acting as powerful regulators of gene expression118. Consequently, understanding the mechanisms behind the dysregulation of miRNAs in various diseases is crucial, as these miRNAs and their targets offer significant therapeutic potential for developing treatments.
Although miRNA research is crucial, there is a significant gap in the literature regarding miRNA activity in PD. Additionally, the findings from various miRNA studies have been inconsistent. Several potential limitations exist in the published research. One major challenge in identifying clinically significant miRNAs in diseases is the small sample sizes of clinical studies, which may lack the statistical power to detect meaningful differences in effect size. Additionally, the methods employed to characterise the miRNA profile in each study vary in sensitivity, influencing the resulting data. The absence of a standardised protocol for miRNA isolation and detection has resulted in the identification of distinct and non-replicable sets of potentially dysregulated miRNAs. Variations in study designs, experimental conditions, tissue sources, PD models, and sample characteristics—including sample size, clinical features, and pharmacological treatments—can lead to inconsistent results among studies. Consequently, clinical papers on the same disease might present vastly different miRNA expression profiles, complicating the process of identifying or validating biologically significant miRNAs119,120.
Challenges in miRNA-based therapeutic therapies
Using miRNAs as therapeutic agents presents a considerable challenge due to the difficulty in delivering them across the BBB into the CNS. This barrier restricts the entry of active substances, thus hindering the effectiveness of miRNA transfection. To overcome this obstacle, two approaches have been devised: restoring diminished miRNA levels using miRNA mimics (agonists) and inhibiting miRNA activity with anti-miRs (antagonists) to counteract excessive miRNA function121,122,123,124,125,126,127.
The potential of miRNA-based therapies is significant, due to their potency, effectiveness, ability to silence genes for optimal durations, simplicity, safety, and easier manufacturing. However, there are challenges to overcome. Extracellularly, barriers like low RNA availability, enzymatic breakdown in the bloodstream, quick removal by the kidneys, uptake by immune cells, and toxicity due to immune responses pose obstacles. Intracellularly, like imprecise targeting, inefficient cellular uptake, and ineffective endosomal processing hinder their effectiveness. Addressing these hurdles is crucial for enhancing miRNA therapy’s effectiveness by improving its pharmacokinetic and pharmacodynamic properties124,125.
The uptake of miRNA-based therapeutic agents by unintended tissues can result in potential off-target effects. Additionally, the distribution of various therapeutics in the CNS is influenced by the presence of numerous specific transporters and receptors123,128. Another limitation is the susceptibility of bare nucleic acids to degradation by enzymes before reaching their intended destinations. Hence, a biologically responsive delivery system can shield the nucleic acids from degradation in serum and facilitate their penetration into targeted cells128.
References
-
de Lau, L. M. & Breteler, M. M. Epidemiology of Parkinson’s disease. Lancet Neurol. 5, 525–535 (2006).
-
Jankovic, J. Parkinson’s disease: clinical features and diagnosis. J. Neurol. Neurosurg. Psychiatry 79, 368–376 (2008).
-
Kramer, M. L. & Schulz-Schaeffer, W. J. Presynaptic alpha-synuclein aggregates, not Lewy bodies, cause neurodegeneration in dementia with Lewy bodies. J. Neurosci. 27, 1405–1410 (2007).
-
Hayes, M. T. Parkinson’s disease and parkinsonism. Am. J. Med. 132, 802–807 (2019).
-
Elkouzi, A., Vedam-Mai, V., Eisinger, R. S. & Okun, M. S. Emerging therapies in Parkinson disease — repurposed drugs and new approaches. Nat. Rev. Neurol. 15, 204–223 (2019).
-
Jankovic, J. & Aguilar, L. G. Current approaches to the treatment of Parkinson’s disease. Neuropsychiatr. Dis. Treat. 4, 743–757 (2008).
-
Grassi, D. et al. Identification of a highly neurotoxic α-synuclein species inducing mitochondrial damage and mitophagy in Parkinson’s disease. Proc. Natl Acad. Sci. USA 115, E2634–E2643 (2018).
-
Singh, A., Zhi, L. & Zhang, H. LRRK2 and mitochondria: recent advances and current views. Brain Res. 1702, 96–104 (2019).
-
Brochard, V. et al. Infiltration of CD4+ lymphocytes into the brain contributes to neurodegeneration in a mouse model of Parkinson disease. J. Clin. Invest. 119, 182–192 (2009).
-
Kortekaas, R. et al. Blood–brain barrier dysfunction in parkinsonian midbrain in vivo. Ann. Neurol. 57, 176–179 (2005).
-
Peball, M. et al. Non-motor symptoms in parkinson’s disease are reduced by nabilone. Ann. Neurol. 88, 712–722 (2020).
-
Pang, S. Y. Y. et al. The interplay of aging, genetics and environmental factors in the pathogenesis of Parkinson’s disease. Transl. Neurodegener. 8, 23 (2019).
-
Fujita, K. A. et al. Integrating pathways of Parkinson’s disease in a molecular interaction map. Mol. Neurobiol. 49, 88–102 (2014).
-
Kim, J. et al. A microRNA feedback circuit in midbrain dopamine neurons. Science 317, 1220–1224 (2007).
-
Vallelunga, A. et al. Identification of circulating microRNAs for the differential diagnosis of Parkinson’s disease and Multiple System Atrophy. Front. Cell. Neurosci. 8, 156 (2014).
-
Yang, Z. et al. Altered expression levels of microRNA-132 and Nurr1 in peripheral blood of parkinson’s disease: potential disease biomarkers. ACS Chem. Neurosci. 10, 2243–2249 (2019).
-
Tolosa, E. et al. MicroRNA alterations in iPSC-derived dopaminergic neurons from Parkinson disease patients. Neurobiol. Aging 69, 283–291 (2018).
-
Mo, M. et al. MicroRNA expressing profiles in A53T mutant alpha-synuclein transgenic mice and Parkinsonian. Oncotarget 8, 15–28 (2017).
-
Chi, J. et al. Integrated analysis and identification of novel biomarkers in Parkinson’s disease. Front. Aging Neurosci. 10, 178 (2018).
-
Nair, V. D. & Ge, Y. Alterations of miRNAs reveal a dysregulated molecular regulatory network in Parkinson’s disease striatum. Neurosci. Lett. 629, 99–104 (2016).
-
Xing, R. X., Li, L. G., Liu, X. W., Tian, B. X. & Cheng, Y. Down regulation of miR-218, miR-124, and miR-144 relates to Parkinson’s disease via activating NF-κB signaling. Kaohsiung J. Med Sci. 36, 786–792 (2020).
-
Schlaudraff, F. et al. Orchestrated increase of dopamine and PARK mRNAs but not miR-133b in dopamine neurons in Parkinson’s disease. Neurobiol. Aging 35, 2302–2315 (2014).
-
Chen, Q. et al. Elevated plasma miR-133b and miR-221-3p as biomarkers for early Parkinson’s disease. Sci. Rep. 11, 15268 (2021).
-
Ren, Y., Li, H., Xie, W., Wei, N. & Liu, M. MicroRNA 195 triggers neuroinflammation in Parkinson’s disease in a Rho associated kinase 1 dependent manner. Mol. Med Rep. 19, 5153–5161 (2019).
-
Tétreault, N. & De Guire, V. miRNAs: their discovery, biogenesis and mechanism of action. Clin. Biochem 46, 842–845 (2013).
-
Almeida, M. I., Reis, R. M. & Calin, G. A. MicroRNA history: discovery, recent applications, and next frontiers. Mutat. Res. 717, 1–8 (2011).
-
Schwarzenbach, H. & Gahan, P. B. MicroRNA shuttle from cell-to-cell by exosomes and its impact in cancer. Non-Coding RNA 5, 28 (2019). Page 28 5.
-
Heman-Ackah, S. M., Hallegger, M., Rao, M. S. & Wood, M. J. A. RISC in PD: the impact of microRNAs in parkinson’s disease cellular and molecular pathogenesis. Front. Mol. Neurosci. 6, 40 (2013).
-
Kehl, T. et al. About miRNAs, miRNA seeds, target genes and target pathways. Oncotarget 8, 107167–107175 (2017).
-
Bano, R., Ahmad, F. & Mohsin, M. A perspective on the isolation and characterization of extracellular vesicles from different biofluids. RSC Adv. 11, 19598–19615 (2021).
-
Jahromi, F. N. A., Dowran, R. & Jafari, R. Recent advances in the roles of exosomal microRNAs (exomiRs) in hematologic neoplasms: pathogenesis, diagnosis, and treatment. Cell Commun. Signal 21, 88 (2023).
-
Nail, H. M., Chiu, C. C., Leung, C. H., Ahmed, M. M. M. & Wang, H. M. D. Exosomal miRNA-mediated intercellular communications and immunomodulatory effects in tumor microenvironments. J. Biomed. Sci. 30, 1–23 (2023).
-
Yu, X., Odenthal, M. & Fries, J. W. U. Exosomes as miRNA carriers: formation– function–future. Int. J. Mol. Sci. 17, 2028 (2016).
-
Hu, G., Drescher, K. M. & Chen, X. M. Exosomal miRNAs: biological properties and therapeutic potential. Front. Genet. 3, 22036 (2012).
-
Deng, T. et al. Exosome miR-155 derived from gastric carcinoma promotes angiogenesis by targeting the c-MYB/VEGF axis of endothelial cells. Mol. Ther. Nucleic Acids 19, 1449 (2020).
-
Pei, X., Li, Y., Zhu, L. & Zhou, Z. Astrocyte-derived exosomes transfer miR-190b to inhibit oxygen and glucose deprivation-induced autophagy and neuronal apoptosis. Cell Cylce 19, 906–917 (2020).
-
Kalluri, R. & LeBleu, V. S. The biology, function, and biomedical applications of exosomes. Science 367, eaau6977 (2020).
-
Liew, L. C., Katsuda, T., Gailhouste, L., Nakagama, H. & Ochiya, T. Mesenchymal stem cell-derived extracellular vesicles: a glimmer of hope in treating Alzheimer’s disease. Int. Immunol. 29, 11–19 (2017).
-
Asai, H. et al. Depletion of microglia and inhibition of exosome synthesis halt tau propagation. Nat. Neurosci. 18, 1584–1593 (2015).
-
Valadi, H. et al. Exosome-mediated transfer of mRNAs and microRNAs is a novel mechanism of genetic exchange between cells. Nat. Cell Biol. 9, 654–659 (2007).
-
Ha, D., Yang, N. & Nadithe, V. Exosomes as therapeutic drug carriers and delivery vehicles across biological membranes: current perspectives and future challenges. Acta Pharm. Sin. B 6, 287–296 (2016).
-
Konovalova, J., Gerasymchuk, D., Parkkinen, I., Chmielarz, P. & Domanskyi, A. Interplay between microRNAs and oxidative stress in neurodegenerative diseases. Int J. Mol. Sci. 20, 6055 (2019).
-
Yakimchuk, K. Exosomes: isolation methods and specific markers. Mater Methods 5, 1450 (2015).
-
Lim, J. et al. Direct isolation and characterization of circulating exosomes from biological samples using magnetic nanowires. J. Nanobiotechnol. 17, 1–12 (2019).
-
Mahgoub, E. O. & Abdella, G. M. Improved exosome isolation methods from non-small lung cancer cells (NC1975) and their characterization using morphological and surface protein biomarker methods. J. Cancer Res. Clin. Oncol. 149, 7505–7514 (2023).
-
Chen, J. et al. Review on strategies and technologies for exosome isolation and purification. Front. Bioeng. Biotechnol. 9, 811971 (2022).
-
Valera-Alberni, M. & Canto, C. Mitochondrial stress management: a dynamic journey. Cell Stress 2, 253–274 (2018).
-
Thanan, R. et al. Oxidative stress and its significant roles in neurodegenerative diseases and cancer. Int. J. Mol. Sci. 16, 193–217 (2014).
-
Chang, K. H. & Chen, C. M. The role of oxidative stress in Parkinson’s disease. Antioxidants 9, 1–32 (2020).
-
Dias, V., Junn, E. & Mouradian, M. M. The role of oxidative stress in Parkinson’s disease. J. Parkinsons Dis. 3, 461–491 (2013).
-
Albers, D. S. & Flint Beal, M. Mitochondrial dysfunction and oxidative stress in aging and neurodegenerative disease. J. Neural Transmission https://doi.org/10.1007/978-3-7091-6781-6.16/COVER (2000).
-
Wang, X. et al. The role of exosomal microRNAs and oxidative stress in neurodegenerative diseases. Oxid. Med. Cell Longev. 2020, 3232869 (2020).
-
Olufunmilayo, E. O., Gerke-Duncan, M. B. & Holsinger, R. M. D. Oxidative stress and antioxidants in neurodegenerative disorders. Antioxidants 12, 517 (2023).
-
Chua, C. E. L. & Tang, B. L. miR-34a in neurophysiology and neuropathology. J. Mol. Neurosci. 67, 235–246 (2018).
-
Deshmukh, P., Unni, S., Krishnappa, G. & Padmanabhan, B. The Keap1–Nrf2 pathway: promising therapeutic target to counteract ROS-mediated damage in cancers and neurodegenerative diseases. Biophys. Rev. 9, 41–56 (2016).
-
Ba, Q. et al. Schisandrin B shows neuroprotective effect in 6-OHDA-induced Parkinson’s disease via inhibiting the negative modulation of miR-34a on Nrf2 pathway. Biomed. Pharmacother. 75, 165–172 (2015).
-
Mao, S., Sun, Q., Xiao, H., Zhang, C. & Li, L. Secreted miR-34a in astrocytic shedding vesicles enhanced the vulnerability of dopaminergic neurons to neurotoxins by targeting Bcl-2. Protein Cell 6, 529–540 (2015).
-
Chen, P., Chen, F., Lei, J., Li, Q. & Zhou, B. Activation of the miR-34a-mediated SIRT1/mTOR signaling pathway by urolithin a attenuates d-galactose-induced brain aging in mice. Neurotherapeutics 16, 1269–1282 (2019).
-
Jiang, Y. et al. Serum secreted miR-137-containing exosomes affects oxidative stress of neurons by regulating OXR1 in Parkinson’s disease. Brain Res. 1722, 146331 (2019).
-
Li, N. et al. Plasma levels of miR-137 and miR-124 are associated with Parkinson’s disease but not with Parkinson’s disease with depression. Neurol. Sci. 38, 761–767 (2017).
-
Kong, Y. et al. High throughput sequencing identifies microRNAs mediating α- synuclein toxicity by targeting neuroactive-ligand receptor interaction pathway in early stage of drosophila Parkinson’s disease model. PLoS ONE 10, e0137432 (2015).
-
Asikainen, S. et al. Global microRNA expression profiling of caenorhabditis elegans Parkinson’s disease models. J. Mol. Neurosci. 41, 210–218 (2010).
-
Shamsuzzama, Kumar, L., Haque, R. & Nazir, A. Role of microRNA Let-7 in modulating multifactorial aspect of neurodegenerative diseases: an overview. Mol. Neurobiol. 53, 2787–2793 (2016).
-
Winkler, C. W., Taylor, K. G. & Peterson, K. E. Location is everything: let-7b microRNA and TLR7 signaling results in a painful TRP. Sci. Signal 7, pe14 (2014).
-
Gehrke, S., Imai, Y., Sokol, N. & Lu, B. Pathogenic LRRK2 negatively regulates microRNA-mediated translational repression. Nature 466:7306 466, 637–641 (2010).
-
Shamsuzzama, Kumar, L. & Nazir, A. Modulation of alpha-synuclein expression and associated effects by microRNA let-7 in transgenic C Elegans. Front. Mol. Neurosci. 10, 328 (2017).
-
Li, S., Lei, Z. & Sun, T. The role of microRNAs in neurodegenerative diseases: a review. Cell Biol. Toxicol. 39, 53–83 (2022).
-
Roy, B., Lee, E., Li, T. & Rampersaud, M. Role of miRNAs in neurodegeneration: from disease cause to tools of biomarker discovery and therapeutics. Genes 2022 13, 425 (2022).
-
Ma, Y. M. & Zhao, L. Mechanism and therapeutic prospect of miRNAs in neurodegenerative diseases. Behav. Neurol. 2023, 8537296 (2023).
-
Hussein, M. & Magdy, R. MicroRNAs in central nervous system disorders: current advances in pathogenesis and treatment. Egypt. J. Neurol. Psychiatry Neurosurg. 57, 1–11 (2021).
-
Tan, L., Yu, J. T. & Tan, L. Causes and consequences of microRNA dysregulation in neurodegenerative diseases. Mol. Neurobiol. 51, 1249–1262 (2015).
-
Gaudet, A. D., Fonken, L. K., Watkins, L. R., Nelson, R. J. & Popovich, P. G. MicroRNAs: roles in regulating neuroinflammation. Neuroscientist 24, 221–245 (2018).
-
Etheridge, A., Lee, I., Hood, L., Galas, D. & Wang, K. Extracellular microRNA: a new source of biomarkers. Mutat. Res. 717, 85–90 (2011).
-
Leung, A. K. L. & Sharp, P. A. MicroRNA functions in stress responses. Mol. Cell 40, 205–215 (2010).
-
Shivdasani, R. A. MicroRNAs: regulators of gene expression and cell differentiation. Blood 108, 3646–3653 (2006).
-
Kamal, M., Mushtaq, G. & Greig, N. Current update on synopsis of miRNA dysregulation in neurological disorders. CNS Neurol. Disord. Drug Targets 14, 492–501 (2015).
-
Parisi, C. et al. MicroRNA-125b regulates microglia activation and motor neuron death in ALS. Cell Death Differ. 23, 531–541 (2016).
-
O’Connell, R. M., Rao, D. S., Chaudhuri, A. A. & Baltimore, D. Physiological and pathological roles for microRNAs in the immune system. Nat. Rev. Immunol. 10, 111–122 (2010).
-
Paisán-Ruíz, C. et al. Cloning of the gene containing mutations that cause PARK8- linked Parkinson’s disease. Neuron 44, 595–600 (2004).
-
Alexandrov, P. N., Zhao, Y., Jones, B. M., Bhattacharjee, S. & Lukiw, W. J. Expression of the phagocytosis-essential protein TREM2 is down-regulated by an aluminum- induced miRNA-34a in a murine microglial cell line. J. Inorg. Biochem 128, 267–269 (2013).
-
Briggs, C. E. et al. Midbrain dopamine neurons in Parkinson’s disease exhibit a dysregulated miRNA and target-gene network. Brain Res. 1618, 111–121 (2015).
-
Hoss, A. G., Labadorf, A., Beach, T. G., Latourelle, J. C. & Myers, R. H. microRNA profiles in Parkinson’s disease prefrontal cortex. Front. Aging Neurosci. 8, 36 (2016).
-
Yao, L. et al. MicroRNA-124 regulates the expression of p62/p38 and promotes autophagy in the inflammatory pathogenesis of Parkinson’s disease. FASEB J. 33, 8648–8665 (2019).
-
Yao, L. et al. MicroRNA-124 regulates the expression of MEKK3 in the inflammatory pathogenesis of Parkinson’s disease. J. Neuroinflammation 15, 1–19 (2018).
-
Li, H. et al. MicroRNA‐150 serves as a diagnostic biomarker and is involved in the inflammatory pathogenesis of Parkinson’s disease. Mol. Genet Genom. Med. 8, e1189 (2020).
-
Zhang, J. et al. miR-let-7a suppresses α-Synuclein-induced microglia inflammation through targeting STAT3 in Parkinson’s disease. Biochem. Biophys. Res. Commun. 519, 740–746 (2019).
-
Feng, Y. et al. Effective inhibition of miR-330/SHIP1/NF-κB signaling pathway via miR- 330 sponge repolarizes microglia differentiation. Cell Biol. Int. 45, 785–794 (2021).
-
He, Q., Wang, Q., Yuan, C. & Wang, Y. Downregulation of miR-7116-5p in microglia by MPP+ sensitizes TNF-α production to induce dopaminergic neuron damage. Glia 65, 1251–1263 (2017).
-
Junn, E. et al. Repression of α-synuclein expression and toxicity by microRNA-7. Proc. Natl Acad. Sci. USA 106, 13052–13057 (2009).
-
Zhou, Y. et al. MicroRNA-7 targets Nod-like receptor protein 3 inflammasome to modulate neuroinflammation in the pathogenesis of Parkinson’s disease. Mol. Neurodegener. 11, 1–15 (2016).
-
Sun, Q. et al. MicroRNA-190 alleviates neuronal damage and inhibits neuroinflammation via Nlrp3 in MPTP-induced Parkinson’s disease mouse model. J. Cell Physiol. 234, 23379–23387 (2019).
-
Wang, R. et al. miR-29c-3p inhibits microglial NLRP3 inflammasome activation by targeting NFAT5 in Parkinson’s disease. Genes Cells 25, 364–374 (2020).
-
Li, D. et al. MicroRNA-30e regulates neuroinflammation in MPTP model of Parkinson’s disease by targeting Nlrp3. Hum. Cell 31, 106–115 (2018).
-
Tan, A., Rajadas, J. & Seifalian, A. M. Exosomes as nano-theranostic delivery platforms for gene therapy. Adv. Drug Deliv. Rev. 65, 357–367 (2013).
-
Chen, J. J., Zhao, B., Zhao, J. & Li, S. Potential roles of exosomal micrornas as diagnostic biomarkers and therapeutic application in Alzheimer’s disease. Neural Plast. 2017, 7027380 (2017).
-
Paul, P. et al. Interplay between miRNAs and human diseases. J. Cell Physiol. 233, 2007–2018 (2018).
-
Hornung, S., Dutta, S. & Bitan, G. CNS-derived blood exosomes as a promising source of biomarkers: opportunities and challenges. Front. Mol. Neurosci. 13, 38 (2020).
-
Lee, S.-T. et al. Exosome-based delivery of miR-124 in a Huntington’s disease model. J. Mov. Disord. 10, 45–52 (2017).
-
Boon, R. A. & Vickers, K. C. Intercellular transport of microRNAs. Arterioscler. Thromb. Vasc. Biol. 33, 186–192 (2013).
-
Bayraktar, R., van Roosbroeck, K. & Calin, G. A. Cell-to-cell communication: microRNAs as hormones. Mol. Oncol. 11, 1673–1686 (2017).
-
Morel, L. et al. Neuronal exosomal mirna-dependent translational regulation of astroglial glutamate transporter glt1. J. Biol. Chem. 288, 7105–7116 (2013).
-
Berindan-Neagoe, I. & Calin, G. A. Molecular pathways: microRNAs, cancer cells, and microenvironment. Clin. Cancer Res. 20, 6247–6253 (2014).
-
Men, Y. et al. Exosome reporter mice reveal the involvement of exosomes in mediating neuron to astroglia communication in the CNS. Nat. Commun. 10, 1–18 (2019).
-
Lafourcade, C., Ramírez, J. P., Luarte, A., Fernández, A. & Wyneken, U. MiRNAs in astrocyte-derived exosomes as possible mediators of neuronal plasticity. J. Exp. Neurosci. 10, 1–9 (2016).
-
Cao, X. Y. et al. MicroRNA biomarkers of Parkinson’s disease in serum exosome-like microvesicles. Neurosci. Lett. 644, 94–99 (2017).
-
Lugli, G. et al. Plasma exosomal miRNAs in persons with and without Alzheimer disease: altered expression and prospects for biomarkers. PLoS ONE 10, e0139233 (2015).
-
Gui, Y. et al. Altered microRNA profiles in cerebrospinal fluid exosome in Parkinson disease and Alzheimer disease. Oncotarget 6, 37043–37053 (2015).
-
Riancho, J., Santurtun, A. & Sánchez-Juan, P. Characterization of alzheimer’s disease micro-RNA profile in exosome-enriched CSF samples. Methods Mol. Biol. 2044, 343–352 (2019).
-
Weber, J. A. et al. The microrna spectrum in 12 body fluids. Clin. Chem. 56, 1733–1741 (2010).
-
Liu, C. G., Song, J., Zhang, Y. Q. & Wang, P. C. MicroRNA-193b is a regulator of amyloid precursor protein in the blood and cerebrospinal fluid derived exosomal microRNA-193b is a biomarker of Alzheimer’s disease. Mol. Med. Rep. 10, 2395–2400 (2014).
-
McKeever, P. M. et al. MicroRNA expression levels are altered in the cerebrospinal fluid of patients with young-onset Alzheimer’s disease. Mol. Neurobiol. 55, 8826–8841 (2018).
-
Wei, H. et al. Serum exosomal miR-223 serves as a potential diagnostic and prognostic biomarker for dementia. Neuroscience 379, 167–176 (2018).
-
Yao, Y.-F., Qu, M.-W., Li, G.-C., Zhang, F.-B. & Rui, H.-C. Circulating exosomal miRNAs as diagnostic biomarkers in Parkinson’s disease. Eur. Rev. Med. Pharmacol. Sci. 22, 5278–5283 (2018).
-
Caggiu, E. et al. Differential expression of miRNA 155 and miRNA 146a in Parkinson’s disease patients. eNeurologicalSci 13, 1–4 (2018).
-
Gui, Y. X., Liu, H., Zhang, L. S., Lv, W. & Hu, X. Y. Altered microRNA profiles in cerebrospinal fluid exosome in Parkinson disease and Alzheimer disease. Oncotarget 6, 37043 (2015).
-
Miñones-Moyano, E. et al. MicroRNA profiling of Parkinson’s disease brains identifies early downregulation of miR-34b/c which modulate mitochondrial function. Hum. Mol. Genet. 20, 3067–3078 (2011).
-
McMillan, K. J. et al. Loss of microRNA-7 regulation leads to α-synuclein accumulation and dopaminergic neuronal loss in vivo. Mol. Ther. 25, 2404–2414 (2017).
-
Gennarino, V. A. et al. HOCTAR database: a unique resource for microRNA target prediction. Gene 480, 51–58 (2011).
-
Goh, S. Y., Chao, Y. X., Dheen, S. T., Tan, E. K. & Tay, S. S. W. Role of microRNAs in Parkinson’s disease. Int. J. Mol. Sci. 20, 5649 (2019).
-
Shaheen, N., Shaheen, A., Diab, R. A. & Desouki, M. T. MicroRNAs (miRNAs) role in hypertension: pathogenesis and promising therapeutics. Ann. Med. Surg. 86, 319–328 (2024).
-
Zhang, Y., Wang, Z. & Gemeinhart, R. A. Progress in microRNA delivery. J. Control Release 172, 962–974 (2013).
-
McDermott, A. M., Heneghan, H. M., Miller, N. & Kerin, M. J. The therapeutic potential of microRNAs: disease modulators and drug targets. Pharm. Res. 28, 3016–3029 (2011).
-
Magen, I. & Hornstein, E. Oligonucleotide-based therapy for neurodegenerative diseases. Brain Res. 1584, 116–128 (2014).
-
Li, Z. & Rana, T. M. Therapeutic targeting of microRNAs: current status and future challenges. Nat. Rev. Drug Discov. 13, 622–638 (2014).
-
Bumcrot, D., Manoharan, M., Koteliansky, V. & Sah, D. W. Y. RNAi therapeutics: a potential new class of pharmaceutical drugs. Nat. Chem. Biol. 2, 711–719 (2006).
-
Soifer, H. S., Rossi, J. J. & Sætrom, P. MicroRNAs in disease and potential therapeutic applications. Mol. Ther. 15, 2070–2079 (2007).
-
van Rooij, E. & Kauppinen, S. Development of microRNA therapeutics is coming of age. EMBO Mol. Med. 6, 851–864 (2014).
-
Chen, Y., Zhao, H., Tan, Z., Zhang, C. & Fu, X. Bottleneck limitations for microRNA- based therapeutics from bench to the bedside. Pharmazie 70, 147–154 (2015).
-
Je, G. & Kim, Y. S. Mitochondrial ROS-mediated post-transcriptional regulation of α- synuclein through miR-7 and miR-153. Neurosci. Lett. 661, 132–136 (2017).
-
Fragkouli, A. & Doxakis, E. miR-7 and miR-153 protect neurons against MPP(+)- induced cell death via upregulation of mTOR pathway. Front. Cell. Neurosci. 8, 182 (2014).
-
Gopalai, A. A. et al. LRRK2 G2385R and R1628P mutations are associated with an increased risk of Parkinson’s disease in the Malaysian population. Biomed. Res. Int. 2014, 867321 (2014).
-
Doxakis, E. Post-transcriptional regulation of alpha-synuclein expression by mir-7 and mir-153. J. Biol. Chem. 285, 12726–12734 (2010).
-
Jiang, J., Xiong, R., Lu, J., Wang, X. & Gu, X. MicroRNA-203a-3p regulates the apoptosis of MPP+ induced SH-SY5Y cells by targeting A-synuclein. J. Biomater. Tissue Eng. 10, 838–844 (2020).
-
Zhou, J. et al. miR-103a-3p regulates mitophagy in Parkinson’s disease through Parkin/Ambra1 signaling. Pharmacol. Res. 160, 105197 (2020).
-
Jauhari, A., Singh, T., Mishra, S., Shankar, J. & Yadav, S. Coordinated action of miR- 146a and parkin gene regulate rotenone-induced neurodegeneration. Toxicol. Sci. 176, 433–445 (2020).
-
Cheng, M. et al. MicroRNA-181a suppresses parkin-mediated mitophagy and sensitizes neuroblastoma cells to mitochondrial uncoupler-induced apoptosis. Oncotarget 7, 42274–42287 (2016).
-
Di Rita, A. et al. miR-218 inhibits mitochondrial clearance by targeting PRKN E3 ubiquitin ligase. Int. J. Mol. Sci. 21, 355 (2020).
-
Liang, C. et al. MicroRNA-140 silencing represses the incidence of Alzheimer’s disease. Neurosci. Lett. 758, 135674 (2021).
-
Kim, J. et al. miR-27a and miR-27b regulate autophagic clearance of damaged mitochondria by targeting PTEN-induced putative kinase 1 (PINK1). Mol. Neurodegener. 11, 55 (2016).
-
Krohn, L. et al. Comprehensive assessment of PINK1 variants in Parkinson’s disease. Neurobiol. Aging 91, 168.e1–168.e5 (2020).
-
Cho, H. J. et al. MicroRNA-205 regulates the expression of Parkinson’s disease-related leucine-rich repeat kinase 2 protein. Hum. Mol. Genet. 22, 608–620 (2013).
-
Wu, Q., Xi, D. Z. & Wang, Y. H. MicroRNA-599 regulates the development of Parkinson’s disease through mediating LRRK2 expression. Eur. Rev. Med. Pharmacol. Sci. 23, 724–731 (2019).
-
Geng, L., Zhang, T., Liu, W. & Chen, Y. miR-494-3p modulates the progression of in vitro and in vivo Parkinson’s disease models by targeting SIRT3. Neurosci. Lett. 675, 23–30 (2018).
-
Xiong, R. et al. MicroRNA-494 reduces DJ-1 expression and exacerbates neurodegeneration. Neurobiol. Aging 35, 705–714 (2014).
-
Chen, Y. et al. MicroRNA-4639 is a regulator of DJ-1 expression and a potential early diagnostic marker for Parkinson’s disease. Front. Aging Neurosci. 9, 232 (2017).
-
Lv, R. et al. Rosmarinic acid alleviates inflammation, apoptosis, and oxidative stress through regulating miR-155-5p in a mice model of Parkinson’s disease. ACS Chem. Neurosci. 11, 3259–3266 (2020).
-
Yao, Y. F., Qu, M. W., Li, G. C., Zhang, F. B. & Rui, H. C. Circulating exosomal miRNAs as diagnostic biomarkers in Parkinson’s disease. Eur. Rev. Med Pharmacol. Sci. 22, 5278–5283 (2018).
-
Barbagallo, C. et al. Specific signatures of serum miRNAs as potential biomarkers to discriminate clinically similar neurodegenerative and vascular-related diseases. Cell Mol. Neurobiol. 40, 531–546 (2020).
Acknowledgements
This research was supported by the University of South Florida (USF) seed grant and the support of the Department of Neurosurgery and Brain Repair, USF.
Author information
Authors and Affiliations
Contributions
Nour Shaheen: conceptualisation, methodology, writing- original draft preparation, and writing- reviewing and editing. Ahmed Shaheen: writing- reviewing and editing. Mahmoud Osama, Abdulqadir J. Nashwan: writing- reviewing and editing. Vishal Bharmauria and Oliver Flouty: writing- reviewing and editing. All authors approved the final manuscript.
Corresponding author
Ethics declarations
Competing interests
The authors declare no competing interests.
Additional information
Publisher’s note Springer Nature remains neutral with regard to jurisdictional claims in published maps and institutional affiliations.
Rights and permissions
Open Access This article is licensed under a Creative Commons Attribution-NonCommercial-NoDerivatives 4.0 International License, which permits any non-commercial use, sharing, distribution and reproduction in any medium or format, as long as you give appropriate credit to the original author(s) and the source, provide a link to the Creative Commons licence, and indicate if you modified the licensed material. You do not have permission under this licence to share adapted material derived from this article or parts of it. The images or other third party material in this article are included in the article’s Creative Commons licence, unless indicated otherwise in a credit line to the material. If material is not included in the article’s Creative Commons licence and your intended use is not permitted by statutory regulation or exceeds the permitted use, you will need to obtain permission directly from the copyright holder. To view a copy of this licence, visit http://creativecommons.org/licenses/by-nc-nd/4.0/.
About this article
Cite this article
Shaheen, N., Shaheen, A., Osama, M. et al. MicroRNAs regulation in Parkinson’s disease, and their potential role as diagnostic and therapeutic targets. npj Parkinsons Dis. 10, 186 (2024). https://doi.org/10.1038/s41531-024-00791-2
-
Received:
-
Accepted:
-
Published:
-
DOI: https://doi.org/10.1038/s41531-024-00791-2